The malignant phenotype
Authors
MD, PhD, Associate Professor, Head of Laboratory of Experimental Oncology. Department of Molecular Pathology, University of Copenhagen
MD, PhD, DMSc, Science Director. Novo Nordisk A/S, and currently on leave from University of Copenhagen
Much has happened in the field of cancer research and treatment over the last decades, and the knowledge of cancer biology has increased tremendously. Today it is fair to say that cancer is no longer enigmatic, but it is still incredibly complicated.
This review by Lund and Kristjansen presents an update on the current knowledge of the molecular background of cancer and highlights new information about common characteristics of the malignant phenotype. The local tumour microenvironment including blood vessels plays an active role in the development and progression of cancer, by recently discovered mechanisms involving inflammatory cells as well as hypoxia and the interstitial fluid pressure.
The molecular mechanisms underlying the processes of neoplasia and the development of the malignant phenotype are generally well understood today. It is fair to say that cancer is no longer enigmatic, but still extremely complicated. In the midst of the plethora of specific molecular alterations and mechanisms, all now mapped as individual components in cancer development, certain general trends seem to link otherwise scattered information together. In the excellent review by Hanahan & Weinberg (1), six essential alterations in cell-tissue physiology were identified as collective hallmarks of the malignant phenotype, albeit through different mechanisms: Self-sufficiency in growth-signalling, insensitivity to growth-inhibitory signals, evasion of apoptosis, limitless replicative potential, sustained angiogenesis, and tissue invasion. In a more recent, also excellent review by Vogelstein & Kinzler (2), eight different cell biological pathways were identified through which most if not all elements of molecular carcinogenesis converge. Interestingly, all eight pathways are somewhat related to or sensitive to the interaction between cell and microenvironment.
The present review highlights new information about common characteristics of the malignant phenotype with special emphasis on the interactions between cancer cells and their surroundings. But before we start on the epigenetics of cancer, a brief update on current knowledge of the genetic background will be presented.
Cancer as a disorder of genes
The common feature of all cancers is that they are the result of somatic mutations. Hundreds of different gene mutations involved in cancer development have been identified in the past decade. Some are subtle intragenic mutations, others are the result of gross chromosomal rearrangements. Some inherited gene defects, which are almost entirely small discrete lesions affecting only single base pairs, increase the risk of eventual cancer development close to certainty. Yet full-grown cancer is always the result of accumulation of several different somatic mutations in a single original cell. In humans no solitary gene defect can alone transform a normal cell to a malignant tumour cell, whereas more short-lived creatures such as mice, whose median life-expectancy outside the protective environment of a research laboratory is 3 months, can develop malignancy by activation of only 1 – 2 oncogenes.
Many different carcinogenic mutations affect the same central growth regulatory pathways, which by the resulting defects promote the ability of cells to acquire autonomous growth. So differently altered genotypes can lead to the same end result: Fatal defects in growth control. Consequently, the malignant phenotype can be the result of mutations in different genes, and each individual patient tumour is a composite result of a unique combination of genetic alterations. The fact that 1) no single gene mutation can drive the development of cancer in humans, and 2) each patient tumour has a unique gene mutation profile, together confers the major obstacles for cancer therapy directed at the cause of the disease.
Three types of genes are affected in multistage carcinogenesis: Oncogenes, tumour suppressor genes and stability genes.
Oncogenes are genes that are mutated in a way that renders the gene product more active and confer a selective growth advantage to the cell. For example, tyrosine kinase growth factor receptors on the surface on the cell membrane normally transfer binding of a growth factor at the outer surface to a growth signal in the cell. In some cancers the growth factor receptor is mutated in a way that renders the inside signaling independent of growth factor binding. As a result the cell receives constant growth signal, independent of the amount of growth factor in the surroundings. Tumour suppressor genes are mutated in a way that reduces the activity of the gene product. Tumour suppressor genes and oncogenes drive the neoplastic process through stimulation of cell growth and inhibition of cell death and cell cycle arrest. Mutation in the third gene type, the stability genes, has a quite different physiological effect. Their gene products are involved in the repair of accidental gene defects that occur during DNA replication, mitosis or exposure to mutagens. When these repair genes are inactivated, the rate of mutations in all genes goes up, since defects are no longer properly repaired. The genetic instability and increased susceptibility to mutations in tumour cell that follows, results in the continuous development of new mutations. As a consequence clones of tumour cells with different gene profiles and phenotypes develop. At the time a tumour reaches detectable size, heterogeneity is a primary characteristic (Fig. 1A). Each single cancer cell contains mutations in multiple genes, gross chromosomal abnormalities, and widespread changes in its gene expression profile.
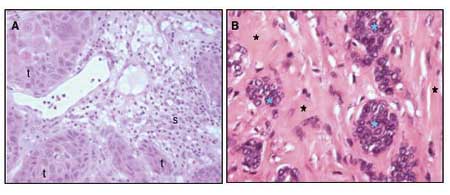
Fig. 1. A: Well-differentiated squamous cell carcinoma from the oral cavity. The morphology of tumour cells (t) is heterogenous. In the stroma (s) surrounding the islets of tumour cells, inflammatory cell infiltration is seen. B: Adenocarcinoma mammae. Islet of tumour cells (blue asterixes) are nested in a collagen-rich stroma (black asterixes).
Tumour microenvironment
Aside from the tumour cells, most solid tumours also consists of varying fractions of different non-malignant stromal cells. These cells participate in the formation of a unique microenvironment and have influence on the malignant behavior of the tumour. An important example is the endothelial cells of tumour blood vessels, which will be further discussed in the angiogenesis section below. Fibroblasts and the collagen-rich extra cellular matrix they produce are often a very prominent part of tumours, as exemplified in breast cancer, where tumour cells most often are nested as small islets of cells in an abundant collagen-rich stroma (Fig. 1B). Activated tumour fibroblasts are not just passive bystanders in the tumourigenic process but can actively participate in the tumourigenesis through their interactions with the tumour cells (3,4). Another important component of the microenvironment is the inflammatory cells. Many cancers arise from sites of infection, chronic irritation and inflammation (5). The degree of chronic inflammatory cell infiltration in tumour tissue is variable between tumour types and from patient to patient, but often a very prominent infiltration with T-lymphocytes and macrophages is seen (Fig. 1A). These inflammatory cells and their chemokines and cytokines, regulate the growth, migration and differentiation of all cell types in the tumour microenvironment, including the tumour cells, fibroblasts, and endothelial cells and also orchestrate changes in the extracellular matrix.
Tumour Associated Macrophages (TAM)
Macrophages are released from the bone marrow as immature monocytes and circulate in the blood before extravasation into tissues where they differentiate into macrophages. Dependent on the local microenvironment, the macrophages develop specific phenotypes. Macrophages perform a multitude of functions essential for tissue remodeling, inflammation and immune responses. Tumour associated macrophages (TAM) have gained considerable interest, both for their pro-tumourigenic action (Fig. 2) as well as for their role as antigen-presenting cells in the cytotoxic immune response to tumour cells. High counts of TAM in patient tumour biopsies are linked to poor prognosis in the majority of studies (6,7), suggesting that the pro-tumour function of macrophages is dominating over their role as immune stimulatory cells in many cancers.
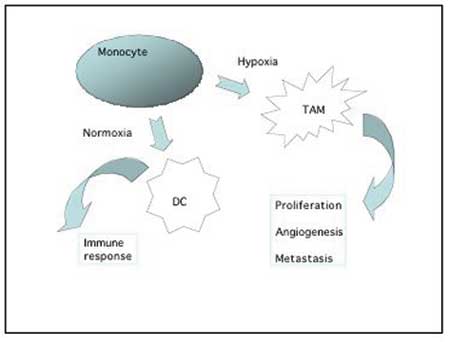
Fig. 2. Monocytes recruited to the tumour environment can either develop into antigen-presenting dendritic cells involved in the immune response against tumour cells, or they can differentiate into pro-tumour macrophages that produce various growth factors, cytokines and proteases which stimulate and support the tumour growth and progression. This type of macrophages are often found accumulating in the hypoxic areas of the tumour.
As support for this concept it has recently been shown that inhibition of macrophage recruitment to tumours can suppress the growth of mammary tumours (8), and that the interaction between tumour cells and macrophages facilitate the migration and invasiveness of tumour cells (7,9).
Macrophages accumulate in hypoxic areas of solid tumours (10), like they accumulate in hypoxic and necrotic areas in other diseases such as atherosclerotic plaques or arthritic joints. In the hypoxic areas the TAM provide a number of trophic functions that promote tumour progression, invasiveness and metastasis. Growth factors and chemokines from TAM provide proliferative and survival signals to tumour cells. Proteases secreted from TAM promote tumour invasion by breaking down basement membranes and remodeling the stromal matrix. This breakdown and remodeling of extracellular matrix components is pivotal for tumour cells to migrate into the surroundings. TAM stimulate angiogenesis in at least three ways: 1) They secrete important angiogenic factors like VEGF and angiopoietins. 2) The proteases produced by TAM are not only necessary for tumour cell migration but also necessary for endothelial cell moving into the tumour tissue as part of the angiogenic process. 3) TAM recruit other hematopoetic cells with similar production of angiogenic factors and proteases, like mast cells and neutrophils – through their production of cytokines. Finally, macrophages can also promote tumourigenesis and tumour progression through their generation of mutagens as by-product of their production of reactive oxygen and nitrogen radicals.
Angiogenesis
In order to grow, solid tumours are dependent on access to blood vessels to acquire an adequate supply of oxygen and nutrients and to remove waste products. In most tumours this access is dependent on the formation of a tumour vasculature by angiogenesis, i.e. formation of new vessels through outgrow from the existing vasculature (11,12). Important exceptions to this rule of angiogenesis-dependence seem to exist; low-malignant astrocytomas can grow by co-opting the existing vasculature, infiltrating the brain along the vessels (13). A minor fraction of non-small cell lung carcinomas showed a clear alveolar vascular pattern with no sign of angiogenesis, likely to represent tumour-exploitation of the existing vasculature (14). Interestingly, in both gliomas and NSCLC the prognosis of the patients with non-angiogenic tumours are better than their angiogenic counterparts. In normal tissue a delicate balance exists between pro-angiogenic factors and anti-angiogenic factors (Fig. 3). At some point of tumour development, a shift towards overweight of pro-angiogenic factors is seen, which leads to sprouting of new vessels from existing ones. In many tumours this is an early event, and angiogenesis is often seen even at a pre-invasive, carcinoma in situ stage of the disease (15). Angiogenic stimulators are produces by both tumour cells and stromal cells. The most potent family of pro-angiogenic factors is the VEGF family (Vascular Endothelial Growth Factor) and VEGFs are crucial for both physiological and pathological angiogenesis (16,17). VEGF is very often upregulated in cancer. This upregulation can happen as a consequence of oncogenic signaling, via hypoxia induced expression, and also via accumulation of VEGF-producing inflammatory cells in the tumour.
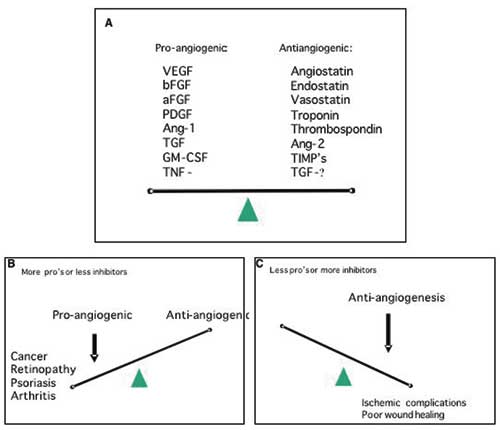
Fig. 3. A: Angiogenesis is regulated by a variety of activators and inhibitors – only a few of which are listed above. In general, the balance between the level of activators and inhibitors dictates whether the vessel endothelium will be in an angiogenic state or quiescent. B: In a number of other diseases besides cancer, excess angiogenesis plays a distinct role in the pathological process. C: Inadequate angiogenesis can be a complicating factor in wound healing and ischemic lesions.
Tumour angiogenesis differs significantly from normal angiogenesis. Normal physiological angiogenesis is a tightly regulated process with a complex interplay between different angiogenic factors, proteases etc. with synergistic effect of some combinations of angiogenic factors, and antagonistic effect of other combinations (18). In tumours the tight regulation of angiogenesis through control of pro-and anti-angiogenic factors, proteases etc. is lost, and the resulting tumour vessels are structurally and functionally abnormal. Loss of the normal hierarchical structure of arterioles, capillaries and venoles is characteristic, and blood vessels are tortuous, dilated or even saccular, and with blind ending buds. Abnormal branching patterns are also seen (Fig. 4). Besides being a pro-angiogenic factor, VEGF is also a permeability factor for endothelial cells, and tumour vessels are often very leaky. In normal tissue angiogenesis the new-formed vessels rapidly mature and become stable. This is not the case in tumours where large fractions of the newly formed vessel are immature and lack the normal pericyte coating. These immature vessels depend on continuous growth factor stimulation in order not to regress, which may explain why anti-angiogenic therapy not only cause decreased angiogenesis but also sometimes lead to vascular regression in tumours (19).
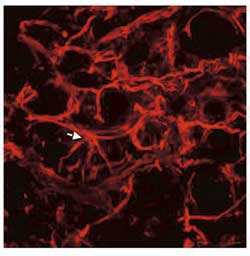
Fig. 4. Blood vessels in an experimental glioblastoma. The vessels are abnormal with a high degree of variation in diameter, they are tortuous, and have an abnormal branching patterns (arrow) as well as blind-ending buds. Lectin-staining, courtesy of Dr. Jørgen Rygaard.
Two important epigenetic characteristics of cancers
As a result of the highly irregular outline of the tumour vasculature, regions of relative or absolute hypoperfusion are predominant in solid tumours. Especially in large bulky tumours is central necrosis frequent. This is generally ascribed to lack of co-ordination between stromal, i.e. vascular, and neoplastic cell growth, leading to chronic hypoxia and ultimate starvation of insufficiently vascularized central parts of the growing tumour. Similarly, on a microscopical level, small necrotic foci are usually scattered around cancer tissues, according to now classic (pre-angiogenesis) theory as a result of the intercapillary distance exceeding twice the diffusion distance of molecular oxygen (20). New insight linking molecular and physiological events regarding tumour hypoxia has placed this phenomenon or characteristic as a key regulator of the malignant phenotype, as will be discussed in more detail below.
Interstitial fluid pressure (IFP)
Another curious effect of the vascular de-arrangement of solid tumours is interstitial hypertension, or increased interstitial fluid pressure (IFP). IFP is the hydrostatic pressure in the interstitial space. In normal tissues this is usually between -1 to 2 mmHg, whereas in a large range of tumours examined in patients as well as in experimental model systems in rodents, values of around 20 mmHg, and sometimes even higher (40 – 60 mmHg), are consistently recorded (21 – 23). Abnormalities in the pressure homeostatic triangular balance between hydrostatic and oncotic pressure gradients and the lymphatic drainage (Fig. 5), adds up to the resultant pan-tumoural increase in IFP (24).
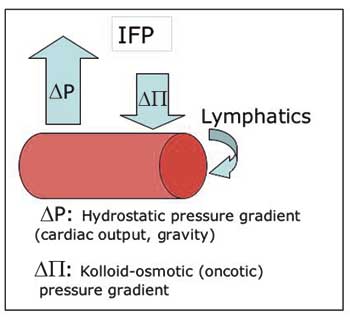
Fig. 5. Starling’s law of filtration: The positive net difference between extravasation by hydrostatic forces and intravasation by osmotic forces is balanced by the lymphatic drainage. Abnormally high interstitial fluid pressure (IFP) can be a result of changes in all three parameters.
The outwardly directed hydrostatic pressure gradient is sensitive to changes in the perfusion pressure, and increases proportional to the increased geometric flow resistance in solid tumours. Blood vessel leakiness account for increased extravasation of macromolecules into the interstitium of solid tumours, resulting in a reduced oncotic gradient. Non-functional tumoural lymphatics fail to level off the net surplus of transvascular fluid transport, which maintains a high pressure equilibrium. Finally, interstitial fibrosis and abnormal contraction of the interstitial matrix by activated stromal myofibroblasts can actively enhance the abnormally high IFP (25, 26). The elevated IFP of solid tumours is associated with important reductions in the uptake of macromolecular agents (26), and to some degree also low-molecular-weight compounds such as paclitaxel and 5-fluoracil (23). The latter is somewhat paradoxical since IFP primarily affects the convective transport, i.e. transport along a pressure gradient which is the principal mechanism for transfer of macromolecules and large particles, whereas small molecules are distributed by diffusion which is relatively independent on IFP. So, the elevated IFP constitutes a key element of physiological resistance of tumours, i.e. lack of efficacy as a result of selectively reduced uptake of otherwise effective therapeutic agents. This is an important resistance mechanism which is distinctly different from the cellular drug resistance mechanisms associated with clonal selection pressure, induction of specific membrane pumps, or adaptive target modulation.
Tumour hypoxia
Two types of hypoxia are found in tumours: Acute, perfusion limited, hypoxia and chronic, diffusion limited hypoxia. Acute hypoxia is the result of transient hypoperfusion, caused by vascular shot down by microthrombi or undue contractions. Chronic hypoxia prevails on the border between normoxic tissues and necrosis, where the oxygen and nutrient supply is just about adequate to sustain cellular life, but not sufficient to permit proliferation and other more complex processes. Such chronically hypoxic cells represent a therapeutic problem due to their relative radioresistance and lack of sensitivity, especially to agents that target proliferating cells. Hypoxic regions thus constitute a sanctuary from which clonogenic cells are recruited following the successful destruction of less hypoxic tumour cells. The adverse microenvironment of solid tumours bring on a general selection pressure towards more robust cellular phenotypes with lesser nutritive demands, and lower vulnerability by changes in the extracellular pH and pO2.
In addition to the indirect shaping of the malignant phenotype by clonal selection in an adverse environment, seminal discoveries during the past decade have taught us how tumour hypoxia plays an active role in modulating the malignant phenotype by governing Hypoxia inducible factor 1 (HIF1), a master regulator of most if not all important genes involved in propagating and maintaining malignancy (2,28).
HIF1 is a transcription factor of mammalian cells. Its prime function is to help the cell survive reduced levels of oxygen, and to some extent also glucose. HIF1 is composed of two subunits, an a-unit and b-unit. HIF1-a is constantly produced and immediately degraded in the cytoplasm, as long as oxygen and VHL (Von Hippel Lindau protein – a classic tumour suppressor gene product) is present. In the absence of oxygen, or when VHL is inactivated, e.g. by mutation, HIF1-a enter the nucleus and combines with HIF1-b, a constitutively expressed nucleic protein. The unified HIF1 induces expression of a plethora of genes all handy for survival in times of severe microenvironmental stress (Fig. 7). Angiogenesis, glycolysis, vasodilatation, and many other processes and factors, as e.g. erythropoietin and loads of cytokines are all up-regulated through HIF1. Even more recently it has become clear that HIF1 is central to all known important pathways in malignancy, including regulation of apoptosis and p53, the very guardian of the genome and of others. In these years many new therapeutics under development have HIF1 and other hypoxia dependent transcription factors as molecular targets. Also some established anticancer drugs act directly of the HIF1 pathway, which impacts their mode of action. This is the case with topothecan, whose antitumour effect is augmented by transient hypoxia, most likely via an inhibitory effect on the HIF (29, 30).
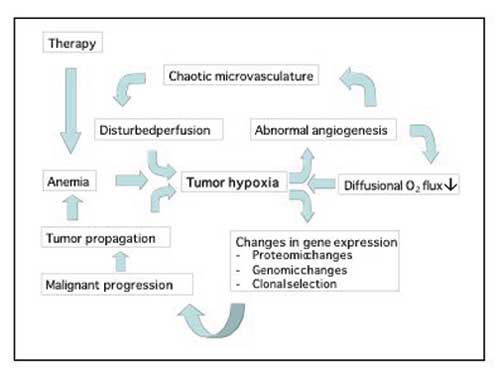
Fig. 6. Tumour hypoxia drives many aspects of the malignant phenotype.
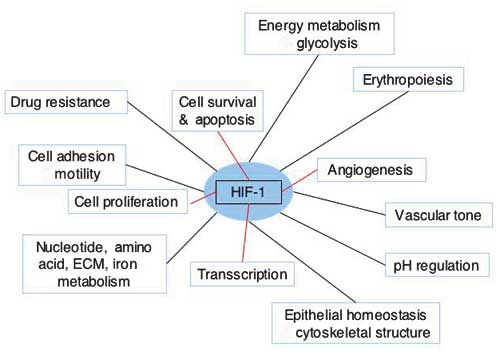
Fig. 7. Cellular functions or functional gene classes regulated by HIF-1. Adapted from Semenza (27).
Other evidence of the dominant role of hypoxia as prime regulator of phenotypical malignancy is persuasively produced by experiments showing increased metastatic potential of tumours, which were subjected to cycles of acute, hypoxic conditions before injection into rodents (31).
References
1. Hanahan D, Weinberg R A. The hallmarks of cancer. Cell 2000; 100: 57 – 70.
2. Vogelstein B, Kinzler, KW. Cancer genes and the pathways they control. Nat Med 2004; 10: 789 – 99.
3. Cunha GR, Hayward SW, Wang YZ. Role of stroma in carcinogenesis of the prostate. Differentiation 2002: 70: 473 – 85.
4. Mueller MM, Fusenig NE. Friends or foes – bipolar effects of the tumour stroma in cancer. Nat Rev Cancer 2004; 4: 839 – 49.
5. Coussens LM, Werb Z. Inflammation and cancer. Nature 2002; 420: 860 – 7.
6. Bingle L, Brown NJ, Lewis CE. The role of tumour-associated macrophages in tumour progression: implications for new anticancer therapies. J Pathol 2002; 196: 254 – 65.
7. Chen JJ, Lin YC, Yao PL, Yuan A, Chen HY, Shun C T, et al. Tumor-associated macrophages: the double-edged sword in cancer progression. J Clin Oncol 2005; 23: 953 – 64.
8. Aharinejad S, Paulus P, Sioud M, Hofmann M, Zins K, Schafer R, et al. Colony-stimulating factor-1 blockade by antisense oligonucleotides and small interfering RNAs suppresses growth of human mammary tumor xenografts in mice. Cancer Res 2004; 64: 5378 – 84.
9. Wyckoff J, Wang W, Lin EY, Wang Y, Pixley F, Stanley ER, et al. A paracrine loop between tumor cells and macrophages is required for tumor cell migration in mammary tumors. Cancer Res 2004; 64: 7022 – 9.
10. Murdoch C, Giannoudis A, Lewis CE. Mechanisms regulating the recruitment of macrophages into hypoxic areas of tumors and other ischemic tissues. Blood 2004; 104: 2224 – 34.
11. Folkman J. Tumor angiogenesis: therapeutic implications. N Engl J Med 1971; 285: 1182 – 6.
12 Bergers G, Benjamin LE. Tumorgenesis and the angiogenic switch. Nat Rev Cancer 2003; 3: 401 – 10.
13. Holash J, Maisonpierre PC, Compton D, Boland P, Alexander CR, Zagzag D, et al. Vessel cooption, regression, and growth in tumors mediated by angiopoietins and VEGF. Science 1999; 284: 1994 – 8.
14. Offersen BV, Pfeiffer P, Hamilton-Dutoit S, Overgaard J. Patterns of angiogenesis in nonsmall-cell lung carcinoma. Cancer 2001; 91: 1500 – 9.
15. Hanahan D, Folkman J. Patterns and emerging mechanisms of the angiogenic switch during tumorigenesis. [Review]. Cell 1996; 86: 353 – 64.
16. Cross MJ, Dixelius J, Matsumoto T, Claesson-Welsh L. VEGF-receptor signal transduction. Trends Biochem Sci 2003; 28: 488 – 94.
17. Lund EL, Thorsen C, Pedersen MW, Junker N, Kristjansen PE. Relationship between vessel density and expression of vascular endothelial growth factor and basic fibroblast growth factor in small cell lung cancer in vivo and in vitro. Clin Cancer Res 2000; 6: 4287 – 91.
18. Ley C, Olsen MB, Lund EL, Kristjansen PE. Angiogenic synergy of bFGF and VEGF is antagonized by angiopoietin-2 in a modified in vivo matrigel assay. Microvasc Res 2004; 68: 161 – 8.
19. Wille CG, Boucher Y, di Tomaso E, Duda DG, Munn L L, Tong RT, et al. Direct evidence that the VEGF-specific antibody bevacizumab has antivascular effects in human rectal cancer. Nature Med 2004; 10: 145 – 7.
20. Thomlinsom RH, Gray LH. The histological structure of some human lung cancers and the possible implications for radiotherapy. Br J Cancer 1955; 9: 539 – 49.
21. Young JS, Lumsden CE, Stalker AL. The significance of the tissue pressure of normal testicular and of neoplastic (Brown-Pearce Carcinoma) tissue in the rabbit. J Pathol Bacteriol 1950; 62: 313 – 33.
22. Jain RK. The next frontier of molecular medicine: Delivery of therapeutics. Nature Med 1988; 4: 655 – 7.
23. Heldin CH, Rubin K, Pietras K, Ostman A. High interstitial fluid pressure – an obstacle in cancer therapy. Nat Rev Cancer 2004; 4: 806 – 13.
24. Boucher Y, Baxter LT, Jain RK. Interstitial pressure-gradients in tissue-isolated and subcutaneous tumors – Implications for therapy. Cancer Res 1990; 50: 4478 – 84.
25. Kristjansen PE. Pathophysiology of human tumor xenografts. Aspects of metabolism, physiology, and pharmacokinetics in heterotransplanted human lung and colon tumors. Dan Med Bull 1997; 44: 380 – 95.
26. Aukland K, Reed RK. Interstitial-lymphatic mechanisms in the control of extracellular fluid volume. Physiol Rev 1993; 73: 1 – 78.
27. Jain RK. Delivery of molecular and cellular medicine to solid tumors. Adv Drug Deliv Rev 2001; 46: 149 – 68.
28. Semenza GL. Targeting HIF-1 for cancer therapy. Nat Rev Cancer 2003; 3: 721 – 32.
29. Lund EL, Hansen LT, Kristjansen PEG. Augmenting tumor sensitivity to topotecan by transient hypoxia. Cancer Chemotherapy Pharmacol 2005; 56: 473 – 480.
30. Rapisarda A, Uranchimeg B, Sordet O, Pommier Y, Shoemaker RH, Melillo G. Topoisomerase I-mediated inhibition of hypoxia-inducible factor 1: mechanism and therapeutic implications. Cancer Res 2004; 64: 1475 – 82.
31. Cairns RA, Hill RP. Acute hypoxia enhances spontaneous lymph node metastasis in an orthotopic murine model of human cervical carcinoma. Cancer Res 2004: 64: 2054 – 61.
Correspondence: Associate Professor, PhD Eva Løbner Lund, Laboratory of Experimental Oncology, Frederik V’s Vej 11, DK-2100 Copenhagen Ø, Denmark. E-mail: eva@pai.ku.dk
Artikkelen har gjennomgått ekstern faglig vurdering.